|
|
|
|
|
Plate 1
When a liquid is heated uniformly from below, it will spontaneously develop
a pattern of hexagonal circulating cells. Here the cells are made visible by metal
flakes suspended in the fluid. (Photo: Manuel Velarde, Universidad Complutense, Madrid.) |
|
|
|
|
|
|
|
|
Plate 2
The rainbow colours of a soap film thinning under
gravity. As the liquid in the film gets pulled downwards,
the film's thickness varies from top to bottom. Interference
between light reflected from the front and back of the film
then selects different wavelengths of reflected light for
different film thicknesses. The film turns silvery and then black
before rupturing. (Photo: Michele Emmer, University of Rome
'La Sapienza'.) |
|
|
|
|
|
|
|
|
Plate 3
Two of the simplest periodic minimal surfaces and their repeat units: (a) the diamond or
D-surface and (b) the gyroid or G-surface. The third member of this family is the P-surface,
shown in Fig. 2.28. (Images: Alan Mackay, Birkbeck College, London.) |
|
|
|
|
|
|
|
|
Plate 4
Turing patterns in a chemical medium. These patterns arise spontaneously through the competition between
a localized autocatalytic chemical reaction and the long-ranged diffusion of a substance that inhibits the reaction.
The colours denote regions of different chemical composition. The patterns are stationary, but a transition from the
stripes to the spots can be induced by changing the composition of the reaction medium. (Images: Harry Swinney,
University of Texas at Austin.) |
|
|
|
|
|
|
|
|
Plate 5
The spectacular patterns of the Belousov-Zhabotinsky reaction consist
of target and spiral waves. (Images: Arthur Winfree, University of Arizona.) |
|
|
|
|
|
|
|
|
Plate 6
The concentric patterns of banded agate are a consequence
of a periodic variation in precipitation of the mineral as it
forms in the Earth. (Photo: Peter Heaney, Princeton University.) |
|
|
|
|
|
|
|
|
Plate 7
Spiral waves in the heart, shown here in a simulation of a
dog heart modelled as an excitable medium. (Image: James
Keener, University of Utah.) |
|
|
|
|
|
|
|
|
Plate 8
Chevron patterns in a colony of the bacterium Escherichia coli grown in a gel. The patterning is a response
to adverse conditions (such as scarcity of nutrients), which induces the bacteria to aggregate through chemical
signalling (chemotaxis). (Photo: Elena Budrene, Harvard University.) |
|
|
|
|
|
|
|
|
Plate 9
Replicating spots in an activator-inhibitor chemical reaction. These spots grow and divide.
When too many are located in one region, they may 'die' through overcrowding.
(Photo: Harry Swinney, University of Texas at Austin.) |
|
|
|
|
|
|
|
|
Plate 10
The characteristic markings on the pelts of great cats (here a Paragyuan jaguar) are produced by
epidermal cells that generate hair-colouring pigments called melanins. |
|
|
|
|
|
|
|
|
Plate 11
Some of nature's most spectacular patterns are found in the multicoloured designs of butterfly wings
(Photo: Fredrick Nijhout, Duke University.) |
|
|
|
|
|
|
|
|
Plate 12
(a) The spectacular 'chiral' growth patterns of a bacterial colony of Bacillus.
(b) The 'vortex' growth mode. Here clumps of bacteria swarm in a blob-like vortex at the tip of each branch.
(Photos: Eshel Ben-Jacob, Tel Aviv University.) |
|
|
|
|
|
|
|
|
Plate 13
Rivers carve out fractal networks, and at the same time they shape the terrain into a rough surface of hills,
mountains and valleys that also has a fractal structure. (Photo: Jim Kirchner, University of California at Berkeley.) |
|
|
|
|
|
|
|
|
Plate 14
Fractal geometry can be used to simulate mountainous topography. (Image: John Beale.) |
|
|
|
|
|
|
|
|
Plate 15
Avalanches in the Earth's mantle are revealed in these fully three-dimensional simulations of mantle convection.
The upwelling (red) and downwelling (blue) regions are shown separately, for clarity. The former are plume-like
structures which rise through the entire mantle. The latter are sheet-like, and become lodged at the 670-km boundary until
catastrophic flushing carries the material through and
down to the base of the mantle. (Images: Paul Tackley, California Institute of Technology, Pasadena.) |
|
|
|
|
|
|
|
|
Plate 16
Freeze-thaw cycles of groundwater at the edge of a lake in Norway produce convection cells that are traced out
by stones on the lake bed. (Photo: Bill Krantz, University of Colorado.) |
|
|
|
|
|
|
|
|
Plate 17
This complex pattern in fluid flow, in which different streamlines (fluid trajectories) are
coloured like the contours of a relief map, arises when a two-dimensional flow
in the vertical direction is driven by a force with fivefold symmetry throughout the plane.
(Image: George Zaslavsky, New York University.) |
|
|
|
|
|
|
|
|
Plate 18
When two dipolar vortices collide, the coherent structures maintain their integrity. The two mushroom-like heads merely
exchange vortices and set off on a new course. Here the dipoles have been coloured with dyes to keep them
identifiable. (Photo: GertJan van Heijst and Jan-Bert Flór, University of Utrecht.) |
|
|
|
|
|
|
|
|
Plate 19
Jupiter's Great Red Spot is an example of a coherent structure in turbulence. It has persisted in Jupiter's swirling
atmosphere for at least 300 years. Other, shorter-lived structures also come and go, like the white spot visible to the
lower right. (Photo: NASA.) |
|
|
|
|
|
|
|
|
Plate 20
Jupiter's banded structure can be mimicked in a laboratory model of the planet, in which
water laced with a fluorescent dye is spun around between an inner and a clear plastic
outer sphere at different temperatures. The bands are due to convective motions
in the fluid. (Photo: Peter Olson, Johns Hopkins University.) |
|
|
|
|
|
|
|
|
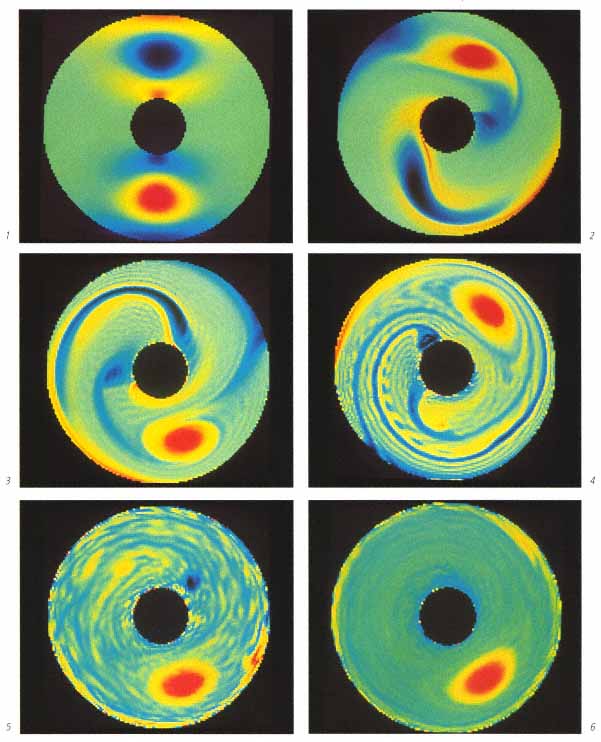 |
|
|
|
|
|
|
|
|
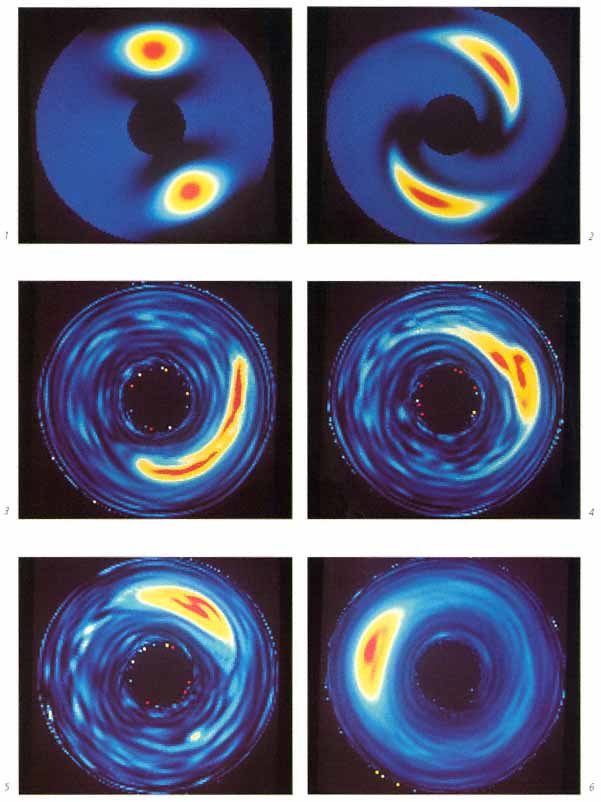 |
|
|
|
|
|
|
|
|
Plate 21
(a) In these computer simulations of atmospheric flow on Jupiter, a hemisphere is projected onto a flat disk
around which the gases rotate. Two vortices are superimposed on the flow (frame 1): one (red) rotating in the same
direction as the mean flow, and the other (blue) rotating in the opposite direction. The colours roughly track the
trajectories that a coloured dye would follow if injected into the vortices. The vortex rotating in the same direction
as the flow remains stable, even though the Reynolds number of the flow is in the turbulent regime. The
counter-rotating vortex, however, is rapidly pulled apart into a spiral, which breaks up via the KelvinHelmholtz
instability into a mass of tiny whorls (you can see some indication of the periodicity of this instability in frame
4). The single remaining vortex, like Jupiter's Great Red Spot, swallows up these little whirlpools, purging them
from the rest of the flow (frame 6).
(b) Two large vortices, both with the same sense of rotation as the mean flow (frame 1), merge into one (frame 6),
implying that a flow containing a single large vortex represents a stable 'attractor' for this system. |
|
|
|
|
|
|
|
|
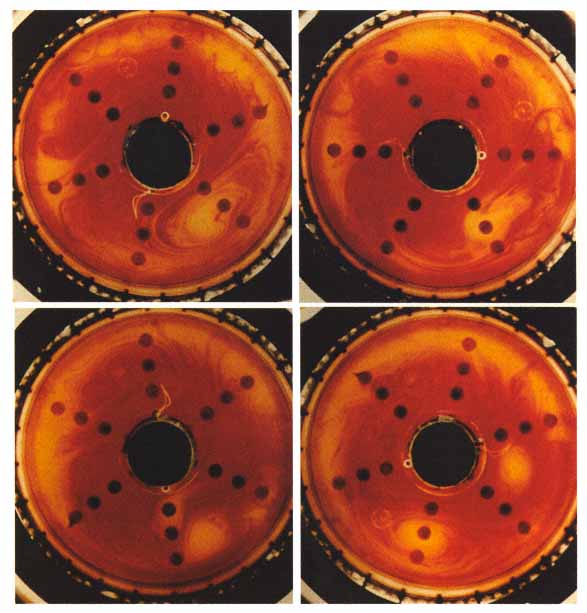 |
|
|
|
|
|
|
|
|
Plate 22
An experimental model of Jupiter's flow in a rotating tank produces a model 'Red Spot' (revealed here by
injecting dye into the fluid), which remains stable despite the turbulence of the flow as a whole. (Photo:
Harry Swinney, University of Texas at Austin.) |
|
|
|
|
|
|
|
|
Plate 23
Sand dunes are an example of a self-organized pattern on a grand scale. (Photo: Jackie Cohen.) |
|
|
|
|
|
|
|
|
Plate 24
The game of cooperation and defection devised by Nowak and May can produce beautiful, symmetric and highly
complex patterns in the two populations of cooperators and defectors. Blue sites represent cooperators that were
cooperators in the last round too, and red sites are defectors that were defectors. Yellow and green sites denote,
respectively, defectors that were cooperators and vice versa; so these sites show where the community boundaries
are changing. These patterns are constantly shifting in a kaleidoscopic manner. (From Nowak and May 1993). |
|
|
|
|